Molecular Orbital Theory
In MO theory, molecular orbitals form by the overlap of atomic orbitals. Atomic orbital energy correlates with electronegativity, as electronegative atoms hold electrons more tightly, lowering their energies. MO modeling is only valid when the atomic orbitals have comparable energy; when the energies differ greatly, the bonding mode becomes ionic. A second condition for overlapping atomic orbitals is that they have identical symmetry.
Two atomic orbitals can overlap in two ways, depending on their phase relationship. An orbital's phase is a direct consequence of electrons' wave-like properties. In graphical representations of orbitals, the orbital phase is depicted either by a plus or minus sign (with no relationship to electric charge) or by shading one lobe. The sign of the phase itself does not have physical meaning except when mixing orbitals to form molecular orbitals. Two same-sign orbitals have a constructive overlap, forming a molecular orbital with the bulk of the electron density located between the two nuclei. This MO is called the bonding orbital, and its energy is lower than that of the original atomic orbitals.
Molecular Orbitals and Symmetry
A bond involving molecular orbitals that are symmetric with respect to rotation around the bond axis is called a sigma bond (σ-bond). If the phase changes, the bond becomes a pi bond (π-bond). Symmetry labels are further defined by whether the orbital maintains its original character after rotating about its center: if so, it is defined gerade, g; if the orbital does not maintain its original character, it is ungerade, u.
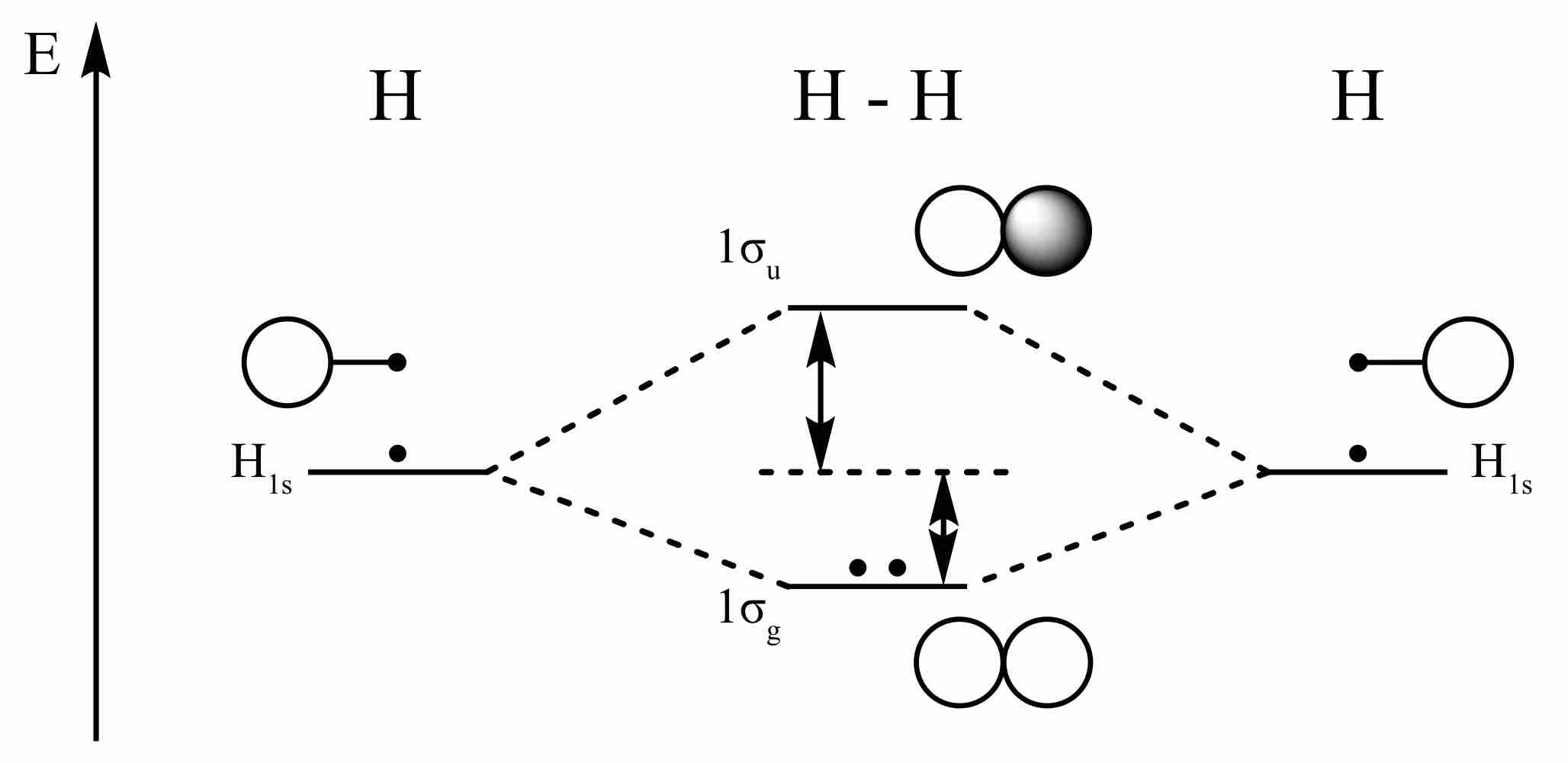
Hydrogen molecule
Bonding and antibonding levels in the hydrogen molecule; the two electrons in the hydrogen atoms occupy a bonding orbital that is lower in energy than the two separate electrons, making this an energy-favorable event.
Atomic orbitals can also interact with each other out-of-phase, leading to destructive cancellation and no electron density between the two nuclei. In this anti-bonding MO, with energy much higher than the original AOs, any electrons present are located in lobes pointing away from the central internuclear axis. For a corresponding σ-bonding orbital, such an orbital would be symmetrical, but are differentiated from it by an asterisk, as in σ*. For a π-bond, corresponding bonding and antibonding orbitals would not have such symmetry around the bond axis, and are designated π and π* respectively.
Filling Electrons in MO Diagrams
The next step in constructing an MO diagram is filling the newly formed molecular orbitals with electrons. Three general rules apply:
- The Aufbau principle states that orbitals are filled starting with the lowest energy
- The Pauli exclusion principle states that the maximum number of electrons. occupying an orbital is two, with opposite spins.
- Hund's rule states that when there are several MOs with equal energy, and the electrons occupy the MOs one at a time before two occupy the same MO.
The filled MO that is highest in energy is called the Highest Occupied Molecular Orbital, or HOMO; the empty MO just above it is the Lowest Unoccupied Molecular Orbital, or LUMO. The electrons in the bonding MOs are called bonding electrons, and any electrons in the antibonding orbital are called antibonding electrons. The reduction these electrons' energy is the driving force for chemical bond formation.
Whenever symmetry or energy make mixing an atomic orbital impossible, a non-bonding MO is created; often quite similar to and with energy levels equal or close to its constituent AO, the non-bonding MO creates an unfavorable energy event. The resulting electron configuration can be described in terms of bond type, parity, and occupancy; one example is dihydrogen (H2): 1σg2. Sometimes, the letter n designates a non-bonding orbital. The presence of a filled antibonding orbital, after fulfilling the conditions above, indicates that the bond in this case does not exist.
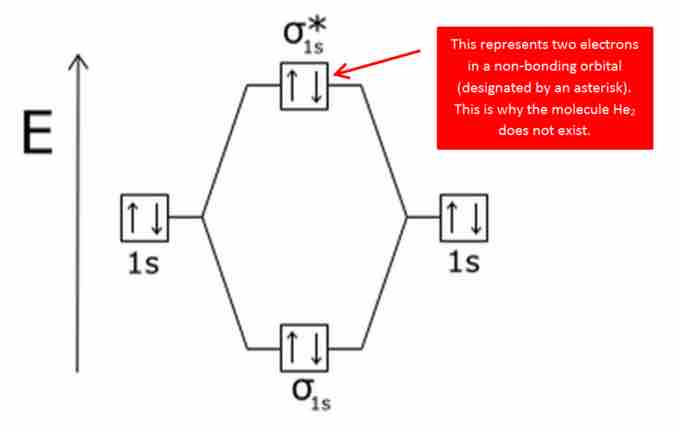
The bonding diagram for the hypothetical molecule He2.
Notice the two electrons occupying the antibonding orbital, which explains why the He2 molecule does not exist.